Abstract
Introduction: Mesenchymal stem cell (MSC) transplantation has been reported as a promising therapy for acute limb ischemia (ALI). However, the treatment efficacy is limited to only certain improvements. Therefore, this study aims to improve the treatment efficacy of MSC transplantation through the use of MSC sheets produced from MSCs' cultured in fibrin scaffold (Fi-MSCs) in ALI models.
Methods: MSCs were isolated and expanded from human umbilical cord tissue. The fibril scaffold was produced from human umbilical cord blood. Fi-MSCs were prepared by mixing MSCs with fibril according to a published protocol, and the Fi-MSC sheets were implanted directly into ligated and transected sites in the hind limbs of ischemic models (treatment group — group I). The results were compared with that of the control group (group II) in which mice were injected with saline. The treatment efficacy was recorded and evaluated through the following assays: limb morphology, SpO2, blood perfusion, angiogenesis, and histological morphology on days 7, 14, and 28 after treatment.
Results: The results indicate that the transplantation of Fi-MSC sheets positively affected the acute ischemia hind-limb mouse models. On day 7 post-transplantation, the SpO2 index recorded at feet in group I (treatment) significantly increased from 79.24% + 1.43% to 89.40% + 1.65% (p-value < 0.05), while in group II (control), the SpO2 index slightly increased from 76.52% + 1.63% to 77.00 + 1.15 (p-value > 0.05). Besides, there were 60.00% (auto-recovery), 13.33%, and 26.67% mice at damage grades 0, I, and II, respectively, in the control group compared to 80%, 20%, and 0% mice at damage grades 0, I, and II, respectively. Moreover, in group I, all mice showed improved blood reperfusion, neovascular, and repaired muscle tissue compared to group II.
Conclusion: Fi-MSC sheet transplantation positively reduced injury and improved blood perfusion ALI in the Swiss mouse model.
Introduction
Peripheral artery disease (PAD) is commonly associated with atherosclerosis that limit blood supply to both the upper and lower extremities, often affecting the lower extremities more severely1. PAD is primarily prevalent in middle-aged people, with a rate of about 20%1. Acute hind-limb ischemia is one of the diseases associated with PAD. Critical limb ischemia is described as a sudden decrease in blood flow to the limb. It can result in amputation or mortality if not treated appropriately2. Approximately 1–1.5 individuals per 10,000 people develop acute limb ischemia (ALI) every year2. Therefore, extensive research is required to improve the effectiveness of treatment.
The causes of ALI can be divided into two groups: traumatic (5%) and nontraumatic (95%). The causes of nontraumatic ALI include arterial embolism (30%), plaque-induced arterial thrombosis (40%), popliteal aneurysm thrombosis (5%), and graft thrombosis (20%)3, 4.
Symptoms of ALI develop rapidly within several minutes, hours, or days after occlusion. The disease progression of a patient’s ALI is described by the term “6Ps”: pain, pallor, paralysis, pulse deficit, paresthesia, and poikilothermia3. Additionally, patients experience intermittent pain to pain at rest, paresthesia, muscle weakness, paralysis, and even gangrene.
Most of the therapies used for ALI are based on thrombolysis, such as streptokinase, saline jets, ultrasound waves, surgical treatment, or intravascular therapy5. Both saline jets and ultrasound waves are used to lyse blood clots. Saline jets can dislodge the blood clot through the Bernoulli effect, while ultrasonic waves can cause physical fragmentation of the blood clot4. Surgical revascularization is administered immediately to eliminate ischemia as soon as possible in patients identified with the severity classification. Intravascular therapy is an initial therapy option for a low level because they have more treatment time2.
In the recent years, stem cell therapy has been considered a promising approach for the treatment of ALI. It shows significant potential in the treatment of ischemia by angiogenesis at ischemic sites1.
Angiogenesis has become a new focus for the treatment of limb ischemia. Angiogenesis reestablishes blood and nutrients supply to the ischemic area, reducing ischemic severity6, 7. Proangiogenic cytokines such as vascular endothelial growth factor (VEGF) and basic fibroblast growth factor (bFGF) are crucial in promoting blood vessel formation and increase collateral circulation in ischemia patients5. Moreover, hUC-MSCs are also known to secrete large amount of cytokines because of the growth factors (GFs) such as VEGF and FGF-2. hUC-MSCs have been proven to promote angiogenesis in vivo and in vitro and have high expression levels of VEGF-1α, MMP-9, VEGFR, and HIF-1α8. To summarize, UCMSCs contribute significantly to the regeneration of blood vessels and reduce the symptoms of ischemic limb8.
Fibrin is an important component responsible for homeostasis and is used as a biopolymer scaffold in tissue engineering. Fibrin also has a key role in angiogenesis9. During wound healing, fibrin provides a suitable environment for angiogenesis. Therefore, the blood supply is restored10, 11. The structure and characteristics of fibrin can trigger the formation of new blood vessels in the wound. Biodegradable substrates (matrix) such as fibrin are considered ideal for tissue engineering applications because they can enable complete tissue regeneration and leave no trace of the original scaffold. The fibrin matrix has widely been used to research blood vessels for tissue engineering and the treatment of ischemic disease. Fibrin’s flexibility in supporting angiogenesis has led it to become an ideal substrate for researching angiogenesis in the body12.
Therefore, this study aims to evaluate the treatment efficacy of mesenchymal stem cell (MSC) sheet transplantation from umbilical-cord-derived MSCs and fibril scaffolds in acute hind-limb ischemic mouse models.
Methods
Animals and samples
In this study, all procedures and manipulations on animals were approved by the Animal Welfare Committee of the Stem Cell Institute, University of Science, VNU-HCM, Vietnam. Swiss six-month-old, male healthy mice were used to establish the acute hind-limb ischemic models.
Umbilical cord tissues and blood samples were collected at hospitals after birth with consent forms. These samples were used for research with the approval of mothers and the hospital ethical committee (District 2, Ho Chi Minh City, VN). All samples were screened for HIV-1/2, HBV, HCV, and CMV. Only samples negative with all these viruses were used in the study.
Culture and expansion of MSCs
MSCs from the human umbilical cord were isolated as per the published protocols13, 14, 15. Briefly, the umbilical cord tissues were collected from the donor with the consent form and the approval of the hospital ethical committee (District 2 General Hospital, Ho Chi Minh City, VN). The tissues were washed twice with a washing buffer solution added with antibiotic-mycotic. Then they were dissected into small fragments (1–2 mm2) and placed into the T-75 flasks with 5 mL of MSCCult I primary medium. These flasks were incubated at 37℃ with 5% CO2 for 5 days. When the migrated cells from the tissues got 70% confluence, they were subcultured to new flasks and discarded tissues. The MSC candidates were expanded and subcultured to the third passage using the MSCCult I medium before they were characterized as MSCs according to the minimal criteria suggested by ISCT16. All chemicals and media were bought from Regemedlab (Ho Chi Minh City, VN).
Characterization of MSC candidates
MSC candidates were characterized as MSCs based on the minimal criteria for MSCs suggested by ISCT in 200616. The expression of MSC-specific marker profile with CD14, CD19, CD34, CD45, CD73, CD90, CD105, and HLA–DR was evaluated. The cells were washed twice with washing buffer (Regenmedlab, Ho Chi Minh City, VN). Subsequently, the cells were stained with the antibodies and incubated at room temperature for 15 minutes in the dark. Then, the cells were washed twice with washing buffer. The stained cells were analyzed using FACSCalibur and BD CellQuest™ Pro software systems (BD Biosciences, Franklin Lakes, NJ).
For in-vitro differentiation assays, the cells were seeded in a 4-well plate with a density of 104 cells/mL with 100 μL of MSCCult I medium for adherence in 48 hours. Then, the media were replaced with suitable differentiation-inducing media for adipogenesis, osteogenesis, and chondrogenesis (bought from Thermo Fisher) for adipocyte, osteocyte, and chondrocyte differentiation, respectively. The cells were cultured for 14 days in the adipogenic medium, 21 days in the chondrogenic medium, and 28 days in the osteogenic medium. The media were refreshed every four days. Finally, the cells were stained with Oil Red (Sigma-Aldrich, USA), Alcian Blue (Sigma-Aldrich, USA), and Alizarin Red (Sigma-Aldrich, USA) to determine their ability to differentiate into adipocytes, chondrocytes, and osteocytes, respectively.
MSC sheet production
MSC sheets (Fi-MSCs) were created by merging MSCs in the fibrin scaffold. The fibrin scaffold was prepared according to a commercial kit’s instruction (5PRP, version U, Regenmedlab, Ho Chi Minh City, VN). Briefly, the umbilical cord blood was transferred to a 50-mL 5PRP tube and centrifuged at 3,000 RPM for 10 minutes. One-third of the upper plasma was removed, and two-third of the lower (platelet-rich plasma – PRP) was collected in a new 50-mL centrifuge tube. Then, 106 MSCs were suspended in 0.5 mL of PRP. The suspension of MSCs and PRP was loaded into a 4-well plate with 0.5 mL per well. Next, 0.12 mL of 0.8 M CaCl2 solution was added to each well.
Acute hind-limb ischemic mouse modeling and Fi -MSC transplantation
Acute hind-limb ischemic mice were used according to the published protocols using 6-month-old healthy mice17, 18, 19. The mice were anesthetized using xylazine (4 mg/kg) and Zoletil (5 mg/kg). Femoral hair was shaved, and then an incision of approximately 1.5 cm long was made in the direction of the femoral blood vessel to open femoral skin at thigh and display femoral veins and arteries.
Both veins and arteries were ligated at two positions at the lateral circumflex artery branches and distally near the popliteal and saphenous artery. The vessels were then cut out at middle of the two ligations. For the treatment group (group I), an Fi-MSC sheet was implanted at the cut site. For the control group, saline was used to wash the cut site. Finally, the skin was stitched, and the wound was coated with povidone-iodine.
Regeneration evaluation of damaged limbs
The degree of ischemic damage at the surgery was recorded, evaluated, and classified according to the guidelines of Goto et al. (2006)20 as follows: grade 0, normal limb without swelling, necrosis, or atrophy of muscle; grade I, necrosis limited to toes; grade II, necrosis extending to the foot; grade III, necrosis extending to the knee; and grade IV: necrosis extending to the femoral region.
Blood flow was assessed indirectly by monitoring the change in oxygen saturation in the blood. This change was detected by the sensor set point in the SpO2 measuring device (Contec 08A, China). Furthermore, blood flow to the hind limbs was also evaluated using trypan blue assay. The 0.04% trypan blue solution was directly injected into the tail vein of the mice. The staining time of the dye that went to the hind limb was recorded based on the blue staining of toes observed by naked eyes.
The formation of new blood vessels was evaluated at 7 days, 14 days, and 28 days after operation. The mice were fixed to the surgical chamber, and the femoral muscle area was assessed. The formation of new blood vessels was observed directly under a stereomicroscope (Zeiss, Germany).
Histological analysis of the limb tissues was also performed at 7 days, 14 days, and 28 days after occlusion. Muscle tissue in hind limbs was biopsied and fixed in 4% PFA for 24 hours. After fixing in PFA, the sample was fixed in a sucrose solution of 30% at 4℃ and until slicing. The samples were cut and stained with hematoxylin and eosin dyes.
Statistical analysis
In this study, VesSeg_V0.1.4 software was used to quantify blood vessels. Data were analyzed by the software GraphPad Prism 8. Statistical analysis was performed by t-test. P-value < 0.05 was considered significant. All data were presented as mean ± standard deviation.
Light microscope image ofblood vessels before occlusion (A), immediately incision (B); 7 days (D, G), 14days (E, H), and 28 days (F,I) after occlusion of the femoral artery. Measurement ofnew blood vessels formed at 28 days postoperative by using VesSeg_V0.1.4software(C,*** p<0.0001).
Results
Isolation and c haracteri zation of human umbilical cord-derived mesenchymal stem cells
Isolated MSCs adhered to the flask surface and displayed spindle-shaped fibroblastic-like morphology (Figure 1 A). In vitro, the MSC candidates successfully differentiated into osteoblasts (Figure 1 B) that were positive with Alizarin Red staining assay, chondroblasts (Figure 1 C) positive with Alcian Blue, and adipocytes (Figure 1 D) positive with Oil Red.
The MSC candidates expressed all MSC-specific maker profiles suggested by ISCT. Indeed, they were positive with CD73 (99.4%), CD90 (99.07%), and CD105 (98.83%), and negative with CD14 (0.07%), CD19 (0.08%), CD34 (0.24%), CD45 (0.88%), and HLA-DR (0.46%; Figure 1 E).
Production of Fi-MSC sheets
Fi-MSCs were formed as semi-solid after being activated with CaCl2 in 2 minutes on a well culture plate (Figure 2 A-B). These cells were evenly distributed on and in a fibrin scaffold with spherical morphology Figure 2 C).
Treatment efficacy of Fi-MSC sheets on acute hind-limb ischemia mice models
Limb oxyhemoglobin saturation-SpO2
At 30 minutes after occlusion, limb oxygen saturation in mice from both control and treatment groups were significantly dereased compared to normal mice (without surgery of produce models; p < 0.05). Oxyen saturation levels recorded at limbs were 76.53% + 1.63% in the control group mice and 79.24% + 0.79% in treatment group mice compared with 95.67% + 0.29% in normal mice. From day 7 to day 14, the SpO2 index recorded at limbs in the control group mice slightly increased from 77.00% + 1.15% to 82.97% + 1.84% and significantly increased from 89.40% +1.00% to 92.60% + 0.71% in treatment group mice (p < 0.05). After 28 days, oxygen saturation recorded in the limbs of both treated and control mice that acquired auto-recovery returned to the normal level (96.93% + 0.88%, 96.67% + 0.96 %, 96.73% + 0.67 %, respectively, for normal mice, treated mice, and auto-recovered control mice) (Figure 3).
Blood flow to the feet
At 7 days after femoral occlusion, it took 185 + 10 s in treated mice (Figure 4 A and 4D) compared with 490 + 127 s in control mice (Figure 4 B and 4D) to for the trypan blue to reach from the tail vein to limb (p < 0.05) and significantly longer in normal mice 77.33 + 1.78 s (p < 0.05, Figure 4 C and 4D).
New blood vessel formation
After 7 days of occlusion, new, small blood vessels appeared at the ligation sites (Figure 5D&G). After 14 days of treatment, more and larger blood vessels were formed than in the control group (Figure 5E&H). Moreover, a measurement of blood vessels using VesSeg_V0.1.4 software revealed that the total area of new vessels per field in the treatment group was 11.41% + 0.24% (Figure 5F&I) on day 28, which is also larger than that of the control group with 6.76% + 0.59% (Figure 5C).
Histological a ssessment
At 7 days post transplantation, the H&E staining of the muscle tissues at the thighs of mice in both control and treatment groups showed cytoplasmic fragmentation (Figure 6 A and 6D). At 14 days, some new blood vessels, the usual morphology of cells, and muscle fibers were observed in the muscle tissues of treated mice (Figure 6 E). Conversely, the muscle tissue structure collected from the control group mice contained some lesions in the cytoplasm and had lost muscle bundle (Figure 6 B). After 28 days, the muscle tissue structure of both groups (auto-recovered control mice) were similar to the normal structure observed in the normal mice (Figure 6 C and F). However, the muscle bundle structure in the treatment group seemed closer to that in normal mice (Figure 5 G).
Hind-limb m orphology and necrosis grade of mice
At 3 days after surgery, there were significant difference in the percentage of mice with lesions at different grades, including grade 0 (Figure 7 A), grade I (Figure 7 B), and grade II (Figure 7 C) in both control and treatment group . In the control group, there were 46.6%, 33.30%, and 20% mice with limb damage at grades 0, I, and II, respectively compared to 73.33%, 26.27%, and 0% of mice with limb damage grades 0, I, and II respectively in the treatment group (Figure 7 D).
On day 7 post transplantation, there were 60.00% (auto-recovery), 13.33%, and 26.67% mice at damage grades 0, I, and II, respectively, in the control group compared to 80%, 20%, and 0% mice at damage grades 0, I, and II, respectively (Figure 7 D).
Discussion
Acute limb ischemia is one of the most serious peripheral artery diseases. It can lead to amputation or even death. Traditional therapies have many limitations and do not stimulate new blood vessel formation. In the recent years, the umbilical cord-derived mesenchymal stem cells have emerged as a potential raw material for regenerative medicine therapy and showed the effectiveness of angiogenesis and injury recovery.
MSCs can produce GFs such as hepatocyte growth factor (HGF), fibroblast growth factor (FGF), VEGF, and high expression of factors that can regulate hematopoietic cell function such as CXCL12, vascular cell adhesion molecules 1, interleukin-7, angiopoietin-1 (Ang-1), and osteopontin21. These factors are essential to blood vessel formation. Moreover, MSCs also can promote the proliferation and migration of endothelial cells in injured tissue22 and promote angiogenesis through the production of vascular factors in ischemic tissues23. Clinically, Fan et al. (2011) demonstrated that MSC transplantation could promote vascular regeneration and improve and restore blood flow of diabetes patients with lower limb ischemia24.
In this study, isolated MSCs were confirmed as MSCs according to the standard recommended by Dominici et al.16. Moreover, the result showed that MSCs exhibited MSC phenotypes. This result is consistent with published studies14, 25.
The fibrin scaffold used in this study was derived from human umbilical cord blood. The fibrin scaffold contributes to the stimulation of new vascular formation and can support cells to proliferate, differentiate, and secrete cytokines by creating a three-dimensional environment26 so they can release PDGF, TGF-β1, EGF, VEGF, bFGF, and so on27. Moreover, the fibrin scaffold is a highly compatible biological substrate and contains many biological factors that are crucial in angiogenesis. This material is also well suited as a scaffold for the fabrication of new tissues. Therefore, the combination of fibrin and MSC creates a promising new material for ALI. This scaffold will help immobilize the stem cells at the desired location.
Although the femoral artery was excised, the most severe degree of necrosis of the mouse limb was amputation to the knee (grade II) according to the classification of Goto et al.20. The cutting of the femoral blood vessel caused a major disruption of blood flow to the underlying tissues such that trypan blue dye reached the walking pads slower than in normal mice, as when blood flow to the limbs decreases, the oxygen carried by the blood also decreases and A significant reduction in blood flow occurs within 1 hour of vascular ablation. However, the collateral system may be activated to reestablish perfusion. After 48 hours, reperfusion to the extremities was enhanced, and the rate of blood transport to the hind limbs improved significantly. This improvement was evident on day 728. After 7 days at the vascular extremity, the increase of blood oxygen saturation was similar to that of a previously published study18.
The reduction of oxygen concentration in the blood flow causes a decrease in nutrition supply, thereby causing severe damage to the structure and function of the cells. Moreover, the disruption of blood circulation also leads to the accumulation of cellular waste products at extremely high concentrations, which is toxic to cells. This lead to cells being unable to repair their damage and enter the process of death instead. This can be one of the leading causes of tissue necrosis29.
Fi-MSC sheet transplantation on ischemic hind limb showed significant effect on preventing necrosis of hind limbs. The results showed that transplanted mice had significantly reduced tissue necrosis, quickly recovered their SpO2 index, sped up the blood circulation, and stabilized muscle tissue structure. Although, under hypoxia conditions, endogenous stem cells could be activated to proliferate and could differentiate into functional cells, such as smooth muscle cells or endothelial cells to form blood vessels this accelerate the wound repair process and reduces necrosis. In this case, transplanted MSCs could significantly contribute to this process. They can produce and secrete GFs to attract intrinsic stem cells to repair tissues, thereby accelerating wound healing30, 31. Factors secreted by MSCs may include exosomes with signaling roles for endothelial cell recruitment and proliferation, promoting early events of angiogenesis, and thereby enhancing reperfusion at the injured area22. MSCs also can secrete GFs such as HGF, FGF, VEGF, and high expression of hematopoietic regulatory factors such as CXCL12, vascular cell adhesion molecule 1, interleukin-7, and Ang-116, or some cytokines such VEGF-1α, MMP-9, VEGFR, and HIF-1α it8. These are essential factors that promote angiogenesis in vivo and in vitro.
Conclusions
The study primarily showed that Fi-MSC sheets helped effectively treat acute ischemic hind limb in murine models. The MSCs from umbilical cord tissues may significantly contribute to neoangiogenesis in combination with fibril effects. Furthermore, the transplantation of Fi-MSCs significantly reduced tissue necrosis and stimulated the tissue regeneration of injured tissues. These results indicate a new promising therapy to treat acute ischemia. However, further studies with more mice to evaluate the side effects of this therapy are required and the existence of MSCs before this study should be translated to clinical trials.
Abbreviations
ALI: Acute limb ischemia
Fi-MSCs: mesenchymal stem cells seeded- fibrin scaffold
HLA-DR: Human Leukocyte Antigen – antigen D Re- lated
hUC-MSCs: human umbilical cord mesenchymal stem cells
PAD: Peripheral artery disease
SD: Standard Deviation
PRP: Platelet Rich Plasma
SpO2: Saturation of peripheral oxygen
VEGF: fibroblast growth factor
This research is funded by University of Science, VNU-HCM under grant number T2020-30.
Author’s contributions
All authors equally contributed in this work. All authors read and approved the final version of the manuscript for submission.
Funding
University of Science, VNU-HCM under grant number T2020-30.
Availability of data and materials
Data and materials used and/or analyzed during the current study are available from the corresponding author on reasonable request.
Ethics approval and consent to participate
Not applicable.
Consent for publication
Not applicable.
Competing interests
The authors declare that they have no competing interests.
References
-
Jeong
I.H.,
Bae
W.Y.,
Choi
J.S.,
Jeong
J.W.,
Ischemia induces autophagy of endothelial cells and stimulates angiogenic effects in a hindlimb ischemia mouse model. Cell Death Dis.
2020;
11
(8)
:
624
.
View Article PubMed Google Scholar -
Obara
H.,
Matsubara
K.,
Kitagawa
Y.,
Acute limb ischemia. Ann Vasc Dis.
2018;
11
(4)
:
443-448
.
View Article PubMed Google Scholar -
Olinic
D.M.,
Stanek
A.,
Tataru
D.A.,
Homorodean
C.,
Olinic
M.,
Acute limb ischemia: an update on diagnosis and management. J Clin Med.
2019;
8
(8)
:
1215
.
View Article PubMed Google Scholar -
Vu
N.B.,
V
Ngoc-Le Trinh,
L.T. Phi,
N.K. Phan,
P. Van Pham,
Human menstrual blood-derived stem cell transplantation for acute hind limb ischemia treatment in mouse models. In Regenerative Medicine (pp. 205-215). Springer, London..
.
-
Oladipupo
S.,
Hu
S.,
Kovalski
J.,
Yao
J.,
Santeford
A.,
Sohn
R.E.,
VEGF is essential for hypoxia-inducible factor-mediated neovascularization but dispensable for endothelial sprouting. Proc Natl Acad Sci USA.
2011;
108
(32)
:
13264-9
.
View Article PubMed Google Scholar -
Brenes
R.A.,
Jadlowiec
C.C.,
Bear
M.,
Hashim
P.,
Protack
C.D.,
Li
X.,
Toward a mouse model of hind limb ischemia to test therapeutic angiogenesis. J Vasc Surg.
2012;
56
(6)
:
1669-79
.
View Article PubMed Google Scholar -
Hu
G.W.,
Li
Q.,
Niu
X.,
Hu
B.,
Liu
J.,
Zhou
S.M.,
Exosomes secreted by human-induced pluripotent stem cell-derived mesenchymal stem cells attenuate limb ischemia by promoting angiogenesis in mice. Stem Cell Res Ther.
2015;
6
(1)
:
10
.
View Article PubMed Google Scholar -
Yin
C.,
Liang
Y.,
Zhang
J.,
Ruan
G.,
Li
Z.,
Pang
R.,
Pan
X.,
Umbilical Cord-Derived Mesenchymal Stem Cells Relieve Hindlimb Ischemia through Enhancing Angiogenesis in Tree Shrews. Stem cells international.
2016;
:
9742034
.
View Article Google Scholar -
Jockenhoevel
S.,
Zund
G.,
Hoerstrup
S.P.,
Chalabi
K.,
Sachweh
J.S.,
Demircan
L.,
Fibrin gel - advantages of a new scaffold in cardiovascular tissue engineering. Eur J Cardiothorac Surg.
2001;
19
(4)
:
424-30
.
View Article PubMed Google Scholar -
Laurens
N.,
Koolwijk
P.,
de Maat
M.P.,
Fibrin structure and wound healing. J Thromb Haemost.
2006;
4
(5)
:
932-9
.
View Article PubMed Google Scholar -
Zisch
A.H.,
Schenk
U.,
Schense
J.C.,
Sakiyama-Elbert
S.E.,
Hubbell
J.A.,
Covalently conjugated VEGF-fibrin matrices for endothelialization. J Control Release.
2001;
72
(1-3)
:
101-13
.
View Article PubMed Google Scholar -
Ceccarelli
J.,
Putnam
A.J.,
Sculpting the blank slate: how fibrin's support of vascularization can inspire biomaterial design. Acta Biomater.
2014;
10
(4)
:
1515-23
.
View Article PubMed Google Scholar -
Dao
T.T.T.,
Nguyen
C.T.H.,
Vu
N.B.,
Le
H.T.N.,
Nguyen
P.D.N.,
Pham
P. Van,
Evaluation of proliferation and osteogenic differentiation of human umbilical cord-derived mesenchymal stem cells in porous scaffolds. In Tissue Engineering and Regenerative Medicine.
2019;
:
207-220
.
View Article Google Scholar -
Van Pham
P.,
Truong
N.C.,
Le
P.T.,
Tran
T.D.,
Vu
N.B.,
Bui
K.H.,
Isolation and proliferation of umbilical cord tissue derived mesenchymal stem cells for clinical applications. Cell Tissue Bank.
2016;
17
(2)
:
289-302
.
View Article PubMed Google Scholar -
Van Pham
P.,
Bich
N.V.,
Phan
N.K.,
Umbilical cord-derived stem cells (Modulatist TM) show strong immunomodulation capacity compared to adipose tissue-derived or bone marrow-derived mesenchymal stem cells. Biomed Res Ther.
2016;
3
(6)
:
1-10
.
View Article Google Scholar -
Dominici
M.,
Le Blanc
K.,
Mueller
I.,
Slaper-Cortenbach
I.,
Marini
F.,
Krause
D.,
Minimal criteria for defining multipotent mesenchymal stromal cells. The International Society for Cellular Therapy position statement. Cytotherapy.
2006;
8
(4)
:
315-7
.
View Article PubMed Google Scholar -
Van Pham
P.,
A comparison of umbilical cord blood-derived endothelial progenitor and mononuclear cell transplantation for the treatment of acute hind-limb ischemia. Biomed Res Ther.
2014;
1
(1)
:
1-12
.
View Article Google Scholar -
Vu
N.B.,
Phi
L.T.,
Dao
T.T.,
Le
H.T.,
Ta
V.T.,
Pham
P.V.,
Adipose derived stem cell transplantation is better than bone marrow mesenchymal stem cell transplantation in treating hind-limb ischemia in mice. Biomed Res Ther.
2016;
3
(9)
:
46
.
View Article Google Scholar -
Vu
N.B.,
Le
H.T.N.,
Dao
T.T.T.,
Phi
L.T.,
Phan
N.K.,
Allogeneic adipose-derived mesenchymal stem cell transplantation enhances the expression of Angiogenic factors in a mouse acute Hindlimb ischemic model. In Stem Cells: Biology and Engineering.
2017;
:
1-17
.
View Article Google Scholar -
Goto
T.,
Fukuyama
N.,
Aki
A.,
Kanabuchi
K.,
Kimura
K.,
Taira
H.,
Search for appropriate experimental methods to create stable hind-limb ischemia in mouse. Tokai J Exp Clin Med.
2006;
31
(3)
:
128-32
.
PubMed Google Scholar -
Nie
W.B.,
Zhang
D.,
Wang
L.S.,
Growth Factor Gene-Modified Mesenchymal Stem Cells in Tissue Regeneration. Drug Des Devel Ther.
2020;
14
:
1241-56
.
View Article PubMed Google Scholar -
Liew
A.,
O'Brien
T.,
Therapeutic potential for mesenchymal stem cell transplantation in critical limb ischemia. Stem Cell Res Ther.
2012;
3
(4)
:
28
.
View Article PubMed Google Scholar -
Liao
L.,
Cell therapy of critical limb ischemia: A review of preclinical and clinical research in China. Vasc Investig Ther.
2020;
3
(1)
:
21
.
View Article Google Scholar -
Fan
Ms,
SymbolHuman umbilical cord mesenchymal stem cells transplantation improves lower limb ischemia of diabetic rabbits. J Clin Rehabil Tissue Eng Res.
2011;
15
(1)
:
33-6
.
-
Nguyen
P.D.,
Vu
N.B.,
Le
H.T.,
Dao
T.T.,
Gia
L.X.,
Pham
P.V.,
Engineered cartilage tissue from biodegradable Poly (ε-caprolactone) scaffold and human umbilical cord derived mesenchymal stem cells. Biomed Res Ther.
2018;
5
(2)
:
2000-12
.
View Article Google Scholar -
Oprea
W.E.,
Karp
J.M.,
Hosseini
M.M.,
Davies
J.E.,
Effect of platelet releasate on bone cell migration and recruitment in vitro. J Craniofac Surg.
2003;
14
(3)
:
292-300
.
View Article PubMed Google Scholar -
Martín-Solé
O.,
Rodó
J.,
García-Aparicio
L.,
Blanch
J.,
Cusí
V.,
Albert
A.,
Effects of platelet-rich plasma (PRP) on a model of renal ischemia-reperfusion in rats. PLoS One.
2016;
11
(8)
:
e0160703
.
View Article PubMed Google Scholar -
Jia
Y.,
Qin
J.,
Zhi
Z.,
Wang
R.K.,
Ultrahigh sensitive optical microangiography reveals depth-resolved microcirculation and its longitudinal response to prolonged ischemic event within skeletal muscles in mice. J Biomed Opt.
2011;
16
(8)
:
086004
.
View Article PubMed Google Scholar -
Basbaum
A.I.,
Bautista
D.M.,
Scherrer
G.,
Julius
D.,
Cellular and molecular mechanisms of pain. Cell.
2009;
139
(2)
:
267-84
.
View Article PubMed Google Scholar -
Chen
L.,
Tredget
E.E.,
Wu
P.Y.,
Wu
Y.,
Paracrine factors of mesenchymal stem cells recruit macrophages and endothelial lineage cells and enhance wound healing. PLoS One.
2008;
3
(4)
:
e1886
.
View Article PubMed Google Scholar -
Hamada
Y.,
Gonda
K.,
Takeda
M.,
Sato
A.,
Watanabe
M.,
Yambe
T.,
In vivo imaging of the molecular distribution of the VEGF receptor during angiogenesis in a mouse model of ischemia. Blood.
2011;
118
(13)
:
e93-100
.
View Article PubMed Google Scholar
Comments
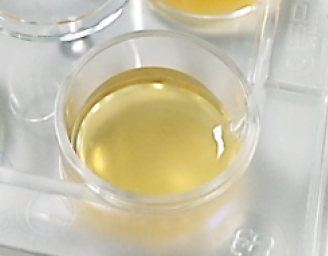
Downloads
Article Details
Volume & Issue : Vol 8 No 6 (2021)
Page No.: 4394-4404
Published on: 2021-06-30
Citations
Copyrights & License

This work is licensed under a Creative Commons Attribution 4.0 International License.
Search Panel
Pubmed
Google Scholar
Pubmed
Google Scholar
Pubmed
Google Scholar
Pubmed
Search for this article in:
Google Scholar
Researchgate
- HTML viewed - 6779 times
- Download downloaded - 1142 times
- View Article downloaded - 0 times